

View larger. | The Large Hadron Collider beauty experiment, aka LHCb. It’s designed to explore what happened after the Big Bang that let matter survive and build the universe we inhabit today. Image via Rosa Menkman/ Flickr
By Patrick Koppenburg, Dutch National Institute for Subatomic Physics and Harry Cliff, University of Cambridge
This month is a time to celebrate. On March 3, 2021, CERN announced the discovery of four brand new particles at the Large Hadron Collider (LHC) in Geneva. This means that the LHC has now found a total of 59 new particles, in addition to the Nobel prize-winning Higgs boson, since it started colliding protons – particles that make up the atomic nucleus along with neutrons – in 2009. Excitingly, while some of these new particles were expected based on our established theories, some were altogether more surprising.
The LHC’s goal is to explore the structure of matter at the shortest distances and highest energies ever probed in the lab – testing our current best theory of nature: the Standard Model of Particle Physics. And the LHC has delivered the goods – it enabled scientists to discover the Higgs boson, the last missing piece of the model. That said, the theory is still far from being fully understood.
One of its most troublesome features is its description of the strong force which holds the atomic nucleus together. The nucleus is made up of protons and neutrons, which are in turn each composed of three tiny particles called quarks (there are six different kinds of quarks: up, down, charm, strange, top and bottom). If we switched the strong force off for a second, all matter would immediately disintegrate into a soup of loose quarks – a state that existed for a fleeting instant at the beginning of the universe.
Don’t get us wrong: the theory of the strong interaction, pretentiously called
“quantum chromodynamics”, is on very solid footing. It describes how quarks interact through the strong force by exchanging particles called gluons. You can think of gluons as analogues of the more familiar photon, the particle of light and carrier of the electromagnetic force.
However, the way gluons interact with quarks makes the strong force behave very differently from electromagnetism. While the electromagnetic force gets weaker as you pull two charged particles apart, the strong force actually gets stronger as you pull two quarks apart. As a result, quarks are forever locked up inside particles called hadrons – particles made of two or more quarks – which includes protons and neutrons. Unless, of course, you smash them open at incredible speeds, as we are doing at CERN.
To complicate matters further, all the particles in the standard model have antiparticles which are nearly identical to themselves but with the opposite charge (or other quantum property). If you pull a quark out of a proton, the force will eventually be strong enough to create a quark-antiquark pair, with the newly created quark going into the proton. You end up with a proton and a brand new “meson”, a particle made of a quark and an antiquark. This may sound weird but according to quantum mechanics, which rules the universe on the smallest of scales, particles can pop out of empty space.
This has been shown repeatedly by experiments – we have never seen a lone quark. An unpleasant feature of the theory of the strong force is that calculations of what would be a simple process in electromagnetism can end up being impossibly complicated. We therefore cannot (yet) prove theoretically that quarks can’t exist on their own. Worse still, we can’t even calculate which combinations of quarks would be viable in nature and which would not.
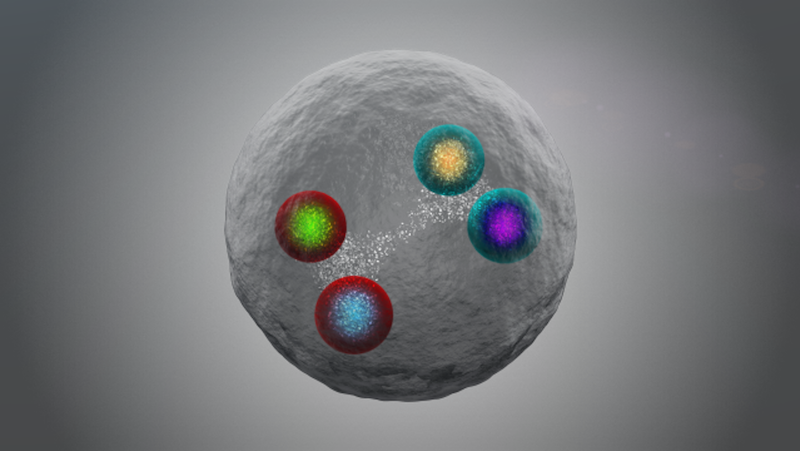
Illustration of a tetraquark. Image via The Conversation.
When quarks were first discovered, scientists realised that several combinations should be possible in theory. This included pairs of quarks and antiquarks (mesons); three quarks (baryons); three antiquarks (antibaryons); two quarks and two antiquarks (tetraquarks); and four quarks and one antiquark (pentaquarks) – as long as the number of quarks minus antiquarks in each combination was a multiple of three.
For a long time, only baryons and mesons were seen in experiments. But in 2003, the Belle experiment in Japan discovered a particle that didn’t fit in anywhere. It turned out to be the first of a long series of tetraquarks. In 2015, the LHCb experiment at the LHC discovered two pentaquarks. The four new particles we’ve discovered recently are all tetraquarks with a charm quark pair and two other quarks. All these objects are particles in the same way as the proton and the neutron are particles. But they are not fundamental particles: quarks and electrons are the true building blocks of matter.
Charming new particles
The LHC has now discovered 59 new hadrons. These include the tetraquarks most recently discovered, but also new mesons and baryons. All these new particles contain heavy quarks such as “charm” and “bottom”.
These hadrons are interesting to study. They tell us what nature considers acceptable as a bound combination of quarks, even if only for very short times. They also tell us what nature does not like. For example, why do all tetra- and pentaquarks contain a charm-quark pair (with just one exception)? And why are there no corresponding particles with strange-quark pairs? There is currently no explanation.
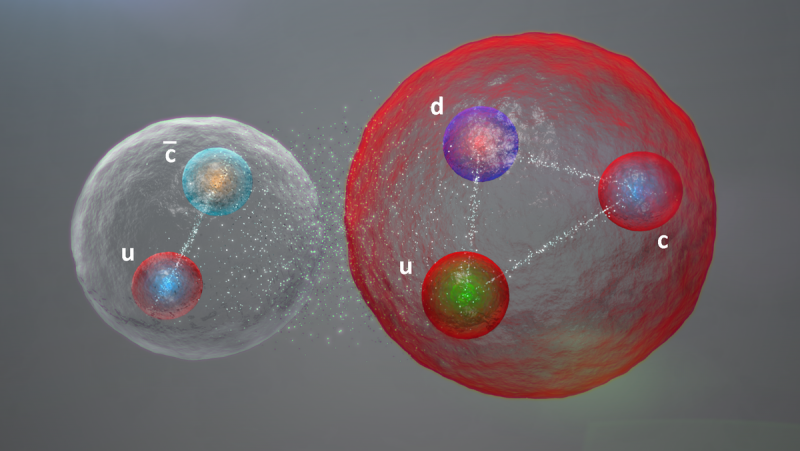
Are pentaquarks molecules? Image via The Conversation.
Another mystery is how these particles are bound together by the strong force. One school of theorists considers them to be compact objects, like the proton or the neutron. Others claim they are akin to “molecules” formed by two loosely bound hadrons. Each newly found hadron allows experiments to measure its mass and other properties, which tell us something about how the strong force behaves. This helps bridge the gap between experiment and theory. The more hadrons we can find, the better we can tune the models to the experimental facts.
These models are crucial to achieve the ultimate goal of the LHC: find physics beyond the standard model. Despite its successes, the standard model is certainly not the last word in the understanding of particles. It is for instance inconsistent with cosmological models describing the formation of the universe.
The LHC is searching for new fundamental particles that could explain these discrepancies. These particles could be visible at the LHC, but hidden in the background of particle interactions. Or they could show up as small quantum mechanical effects in known processes. In either case, a better understanding of the strong force is needed to find them. With each new hadron, we improve our knowledge of nature’s laws, leading us to a better description of the most fundamental properties of matter.
Patrick Koppenburg, Research Fellow in Particle Physics, Dutch National Institute for Subatomic Physics and Harry Cliff, particle physicist, University of Cambridge
This article is republished from The Conversation under a Creative Commons license. Read the original article.
Bottom line: Scientists announced the discovery of four brand new particles at the Large Hadron Collider in Geneva.
from EarthSky https://ift.tt/30E11na


View larger. | The Large Hadron Collider beauty experiment, aka LHCb. It’s designed to explore what happened after the Big Bang that let matter survive and build the universe we inhabit today. Image via Rosa Menkman/ Flickr
By Patrick Koppenburg, Dutch National Institute for Subatomic Physics and Harry Cliff, University of Cambridge
This month is a time to celebrate. On March 3, 2021, CERN announced the discovery of four brand new particles at the Large Hadron Collider (LHC) in Geneva. This means that the LHC has now found a total of 59 new particles, in addition to the Nobel prize-winning Higgs boson, since it started colliding protons – particles that make up the atomic nucleus along with neutrons – in 2009. Excitingly, while some of these new particles were expected based on our established theories, some were altogether more surprising.
The LHC’s goal is to explore the structure of matter at the shortest distances and highest energies ever probed in the lab – testing our current best theory of nature: the Standard Model of Particle Physics. And the LHC has delivered the goods – it enabled scientists to discover the Higgs boson, the last missing piece of the model. That said, the theory is still far from being fully understood.
One of its most troublesome features is its description of the strong force which holds the atomic nucleus together. The nucleus is made up of protons and neutrons, which are in turn each composed of three tiny particles called quarks (there are six different kinds of quarks: up, down, charm, strange, top and bottom). If we switched the strong force off for a second, all matter would immediately disintegrate into a soup of loose quarks – a state that existed for a fleeting instant at the beginning of the universe.
Don’t get us wrong: the theory of the strong interaction, pretentiously called
“quantum chromodynamics”, is on very solid footing. It describes how quarks interact through the strong force by exchanging particles called gluons. You can think of gluons as analogues of the more familiar photon, the particle of light and carrier of the electromagnetic force.
However, the way gluons interact with quarks makes the strong force behave very differently from electromagnetism. While the electromagnetic force gets weaker as you pull two charged particles apart, the strong force actually gets stronger as you pull two quarks apart. As a result, quarks are forever locked up inside particles called hadrons – particles made of two or more quarks – which includes protons and neutrons. Unless, of course, you smash them open at incredible speeds, as we are doing at CERN.
To complicate matters further, all the particles in the standard model have antiparticles which are nearly identical to themselves but with the opposite charge (or other quantum property). If you pull a quark out of a proton, the force will eventually be strong enough to create a quark-antiquark pair, with the newly created quark going into the proton. You end up with a proton and a brand new “meson”, a particle made of a quark and an antiquark. This may sound weird but according to quantum mechanics, which rules the universe on the smallest of scales, particles can pop out of empty space.
This has been shown repeatedly by experiments – we have never seen a lone quark. An unpleasant feature of the theory of the strong force is that calculations of what would be a simple process in electromagnetism can end up being impossibly complicated. We therefore cannot (yet) prove theoretically that quarks can’t exist on their own. Worse still, we can’t even calculate which combinations of quarks would be viable in nature and which would not.
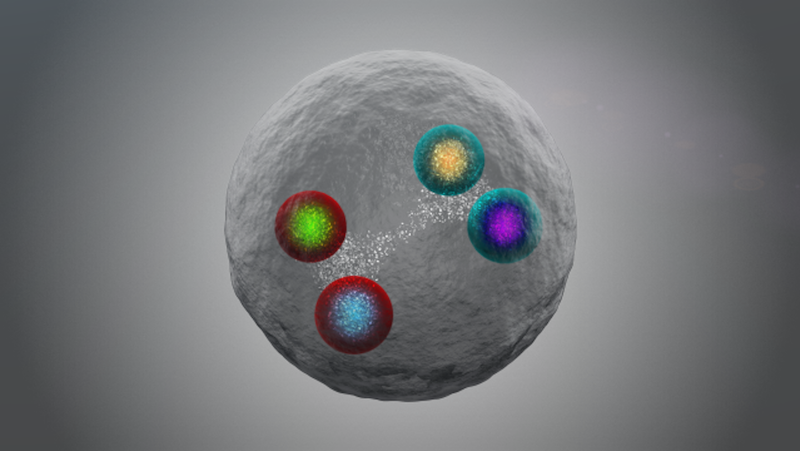
Illustration of a tetraquark. Image via The Conversation.
When quarks were first discovered, scientists realised that several combinations should be possible in theory. This included pairs of quarks and antiquarks (mesons); three quarks (baryons); three antiquarks (antibaryons); two quarks and two antiquarks (tetraquarks); and four quarks and one antiquark (pentaquarks) – as long as the number of quarks minus antiquarks in each combination was a multiple of three.
For a long time, only baryons and mesons were seen in experiments. But in 2003, the Belle experiment in Japan discovered a particle that didn’t fit in anywhere. It turned out to be the first of a long series of tetraquarks. In 2015, the LHCb experiment at the LHC discovered two pentaquarks. The four new particles we’ve discovered recently are all tetraquarks with a charm quark pair and two other quarks. All these objects are particles in the same way as the proton and the neutron are particles. But they are not fundamental particles: quarks and electrons are the true building blocks of matter.
Charming new particles
The LHC has now discovered 59 new hadrons. These include the tetraquarks most recently discovered, but also new mesons and baryons. All these new particles contain heavy quarks such as “charm” and “bottom”.
These hadrons are interesting to study. They tell us what nature considers acceptable as a bound combination of quarks, even if only for very short times. They also tell us what nature does not like. For example, why do all tetra- and pentaquarks contain a charm-quark pair (with just one exception)? And why are there no corresponding particles with strange-quark pairs? There is currently no explanation.
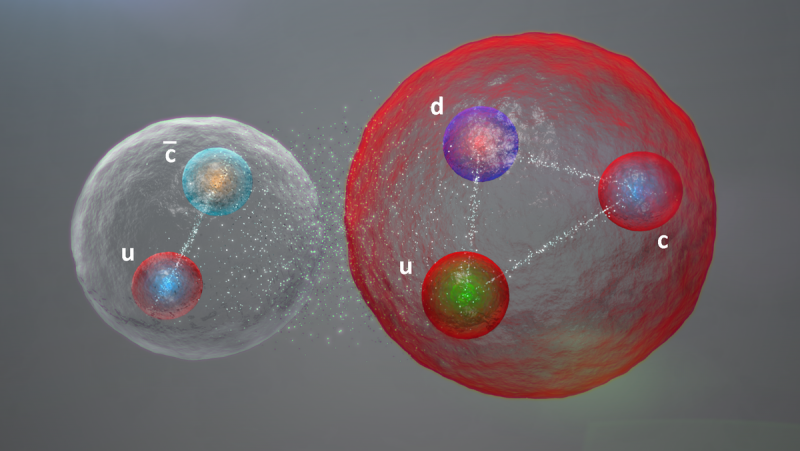
Are pentaquarks molecules? Image via The Conversation.
Another mystery is how these particles are bound together by the strong force. One school of theorists considers them to be compact objects, like the proton or the neutron. Others claim they are akin to “molecules” formed by two loosely bound hadrons. Each newly found hadron allows experiments to measure its mass and other properties, which tell us something about how the strong force behaves. This helps bridge the gap between experiment and theory. The more hadrons we can find, the better we can tune the models to the experimental facts.
These models are crucial to achieve the ultimate goal of the LHC: find physics beyond the standard model. Despite its successes, the standard model is certainly not the last word in the understanding of particles. It is for instance inconsistent with cosmological models describing the formation of the universe.
The LHC is searching for new fundamental particles that could explain these discrepancies. These particles could be visible at the LHC, but hidden in the background of particle interactions. Or they could show up as small quantum mechanical effects in known processes. In either case, a better understanding of the strong force is needed to find them. With each new hadron, we improve our knowledge of nature’s laws, leading us to a better description of the most fundamental properties of matter.
Patrick Koppenburg, Research Fellow in Particle Physics, Dutch National Institute for Subatomic Physics and Harry Cliff, particle physicist, University of Cambridge
This article is republished from The Conversation under a Creative Commons license. Read the original article.
Bottom line: Scientists announced the discovery of four brand new particles at the Large Hadron Collider in Geneva.
from EarthSky https://ift.tt/30E11na
Aucun commentaire:
Enregistrer un commentaire