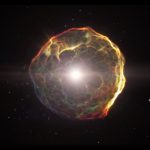
A supernova is the name given to the cataclysmic explosion of a massive star at the end of its life. It can emit more energy in a few seconds than our sun will radiate in its lifetime of billions of years.
The sky above us is strewn with alluringly beautiful remnants of ancient supernovae, that is, stars that lived out their lives and then died in these violent explosions. In a galaxy like our Milky Way, consisting of some 200 billion stars, there should be a supernova as often as every 50 years. Yet supernovae visible to the eye alone are exceedingly rare. You might – or might not – witness one in your lifetime.
EarthSky 2021 lunar calendars now available! They make great gifts. Order now. Going fast!
What we do see are supernova remnants, expanding clouds in space where stars used to be. There are many examples, both inside and outside our galaxy. The most famous supernova remnant visible from the Northern Hemisphere is called the Crab Nebula. It’s located in the direction of the constellation Taurus the Bull. The Chinese recorded witnessing the supernova in the year 1054 CE (although it had occurred 6,523 years earlier, because this star was that many light-years away). They called it a guest star and wrote that it was visible in daylight for a full three weeks, finally fading entirely from view about three months later.
Later, the Crab Nebula became famous for hosting the first known pulsar, discovered in 1967 by Jocelyn Bell Burnell, when she was a graduate student at Cambridge University in England. The Crab pulsar, as it is known, is a neutron star, the remnant of the supernova that created the Crab Nebula. Like cosmic lighthouses, pulsars give off beams of radio waves as they spin. The beams from the Crab pulsar happen to be aimed our way.
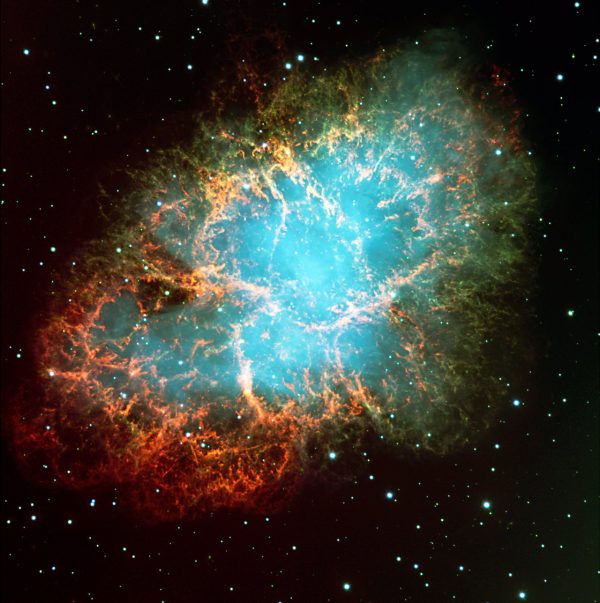
View a larger Crab Nebula image. | This photo shows a 3-color composite of the well-known Crab Nebula (aka Messier 1), as observed via the Hubble Space Telescope in 1999. It is the remnant of a supernova explosion, observed from Earth in the year 1054. It contains a neutron star near its center that spins 30 times per second around its axis. Image via HubbleSite.
So we know that supernovae are star explosions. We see examples of their aftermath in the space around us. We know that – as the star explodes outward – it also implodes, forming an exceedingly dense neutron star that might or might not appear to us on Earth as a pulsar.
But what exactly is a supernova, and what makes it explode?
Astronomers are slowly peeling away the layers of mystery surrounding these exploding stars. Their sheer unpredictability is exciting: each supernova teaches us something new. Astronomers have learned much about supernovae in just the last 50 years. A bright supernova in our Milky Way galaxy is now, statistically, long overdue. Let us hope it happens in our lifetimes, but preferably not too close!
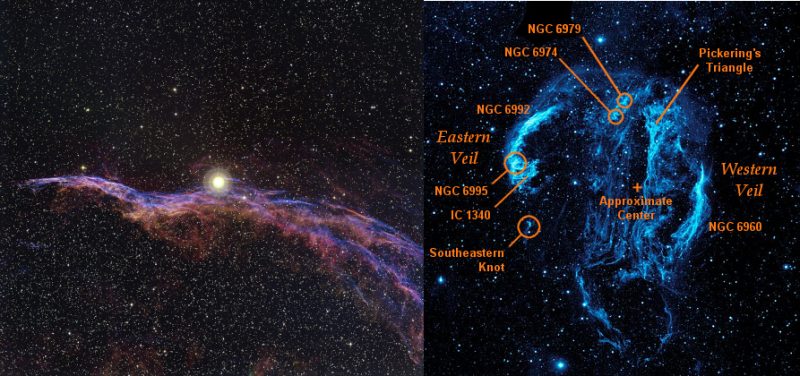
The Veil Nebula in the constellation Cygnus is another famous supernova remnant. It is the visible part of a much larger structure (the Cygnus loop) representing the remains of a star with 40 times the mass of our sun that exploded an estimated 8,000 years ago. Image (left) via T. A. Rector/ University of Alaska Anchorage/ WIYN/ NOAO/ AURA/ NSF and image (right) via NASA/ JPL-Caltech.
A supernova is a more final – and more powerful – explosion than a nova, which is the temporary flaring up of a dwarf star in a binary system. In the nova scenario, the dwarf star collects matter from its companion star. The excess mass causes the dwarf star to flare up suddenly, every now and then, to many times its normal brightness. Then it fades over months to its original brightness before the next flare-up. A supernova, on the other hand, is a much bigger and intrinsically much brighter event (hence the prefix super) where the outer layers of a star are blown explosively into space. A star that goes supernova does not return to its previous brightness and may disappear completely, leaving a an expanding supernova remnant behind.
Both novae and supernovae were once called stella novae (“new stars”), a term coined by the famous Danish astronomer Tycho Brahe in 1572. That is because both novae and supernovae can cause a “new star” to appear in our sky where none was seen before. Both flare up suddenly and – in the case of supernovae – unexpectedly, before slowly fading away entirely over weeks or months.
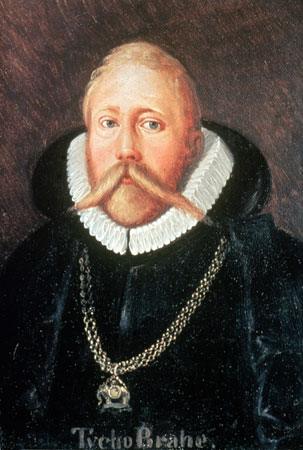
Sixteenth-century astronomer Tycho Brahe coined the term “stella nova” or new star for stars which suddenly brighten, now called novae and supernovae.
Now we know that a supernova is not a new star, but – quite the opposite – an existing one which has reached the end of its life.
And we know that novae – the less powerful flare-ups – mark the location of a star system that might flare up again.
Supernovae can have different causes, but all involve the sudden detonation of a star. Astronomers currently recognize two main types of supernovae, Type I and Type II, according to a way of classifying them devised by German-American astronomer Rudolph Minkowski and Swiss astronomer Fritz Zwicky. Hence the classification system is known as the Minkowski-Zwicky system. The classification is based on the spectra of the supernovae: that is, on their light when it is split into its component colors. Type I supernovae lack the presence of hydrogen in their spectra, while Type II supernovae display it. Type I is further divided up into three subtypes, Ia, Ib and Ic, also based on their spectra.
But maybe more exciting is the difference between the types as determined by the cause of the explosion, and here, a bit confusingly, Types II, Ib and Ic are actually the same kind of explosion, while Type Ia is a completely different creature.
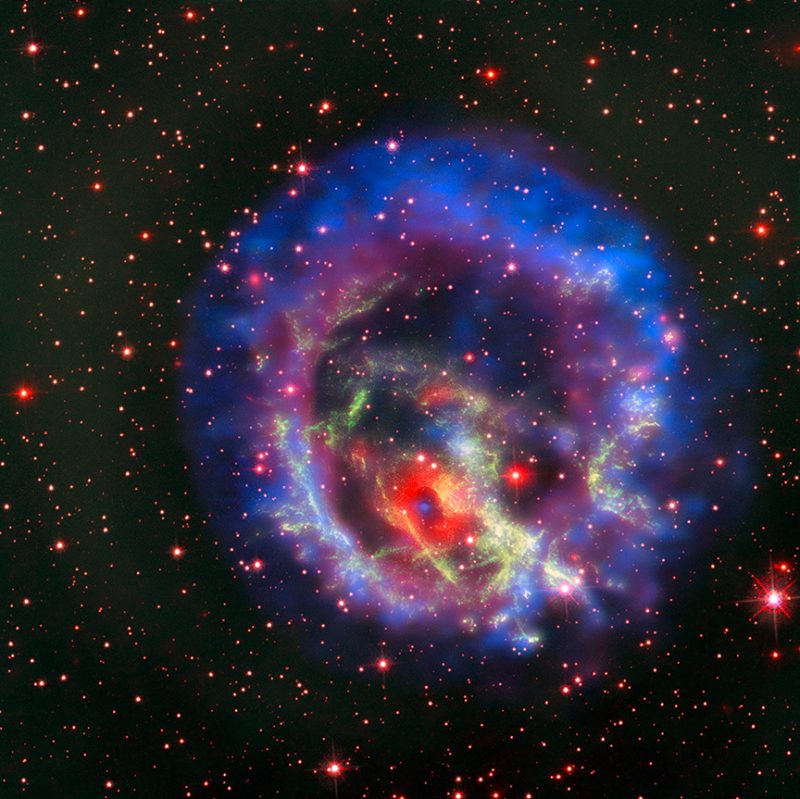
This composite image of the supernova remnant 1E0102.2-7219 contains X-ray emission measured by the Chandra telescope (blue and purple), visible light data from the optical Very Large Telescope’s (VLT) MUSE instrument (bright red), and additional data from the Hubble telescope (dark red and green). A neutron star, the ultra dense core of a massive star that has collapsed in a supernova explosion, is found at its center. Image via NASA Marshall Space Flight Center/ Flickr.
Type II Supernovae
We will start with the more common Type II, which is what people normally think of when they think of a supernova: a star exploding due to old age. Type II supernovae occur when a large star runs out of fuel, which brings it into a rapid collapse and explosion. Such a star is between eight and 40 times heavier than our sun. They are often referred to as “core collapse” supernovae because that’s exactly what happens. The star’s core suddenly – in just a few seconds – collapses in on itself.
But let’s set the scene for that cataclysmic event. For billions of years during this star’s lifetime, nuclear fusion – the process by which hydrogen is converted into helium in the star’s interior, in the process liberating huge amounts of energy, thus enabling the star to shine – had been fighting a battle with gravity. We’re not talking here about the gravitational attraction of one object toward another, but about the star’s own self-gravity. In stars, the outward-pushing radiation from fusion reactions in the star’s core is continually being countered by an inexorable inward-pushing force of gravity. It’s a duel of forces in which neither can be the victor … as long as the nuclear fusion at the core of the star is maintained, the star stays in balance.
But stars are born with a finite amount of hydrogen fuel. After billions of years (around 10 billion in the case of our sun, although our sun isn’t massive enough to produce a supernova), changes begin to take place as hydrogen is depleted. Once the core of the star completely runs out of hydrogen, nuclear fusion in the core ceases; the star has nothing left to “burn.” At that point, the star is no longer able to maintain its outward push against inward-pulling gravity. The star slowly starts to shrink. This shrinking has the effect of bringing more hydrogen from locations further out in the star into the region previously occupied by the core, sufficient hydrogen, in fact, for the nuclear fusion to resume in a shell around the star’s inner core.
However, there is something called the mirror principle, to do with the conservation of gravitational and thermal energy, which states, very simply, that if the core of a star contracts, its outer layers must expand. So the star starts to swell, expanding massively from its original size. As it does so, its outer layers cool, being further from the shell of fusing hydrogen at the core. Once the temperature cools sufficiently, convection rather than radiation becomes the dominant way the outer layers of the star are heated, and the star stops expanding. The star has become a swollen, cooler, and hence reddened, version of its former self; it is now a red giant star.
When our sun becomes a red giant, in about 5 billion years, it will expand to consume – and destroy – Mercury, Venus and possibly Earth too. This means that the sun’s diameter will increase some 115 times; it will also brighten by perhaps 3,000 times.
But – dramatic as its brightness increase may be – we’re not at the supernova stage yet, and reaching the red giant phase is not the end of the story. As the star’s core continues to shrink, temperatures inside it rise to even higher levels than before, reaching a staggering 100 million degrees Celsius. At this extreme temperature, and if the star is massive enough, it can start fusing the next element in the periodic table, that is helium, into carbon, and nuclear fusion starts up once again in its core. Temperatures rise even further, until the helium is used up, leaving a core consisting of carbon and oxygen.
The burning of its helium, however, is not a rapid process: a star eight times the mass of the sun will probably have enough helium to last 100 million years.
However, during all that time, the temperature of the core slowly increases, and when it reaches 500 million degrees, it’s hot enough for the next element to start fusing. Carbon nuclei fuse, producing sodium, neon and magnesium. All of these are burned in turn by the star; the core continues to heat up, reaching 2 billion degrees. As the temperature climbs, first silicon is formed, followed by sulphur, argon, calcium, chromium, manganese and nickel.
Each is burned in successive shells as the core continues to shrink until the star resembles an onion. Each element created in this stellar nucleosynthesis is progressively heavier – consists of larger numbers of protons, neutrons and electrons – until, eventually, one element is produced that cannot be burned: iron.
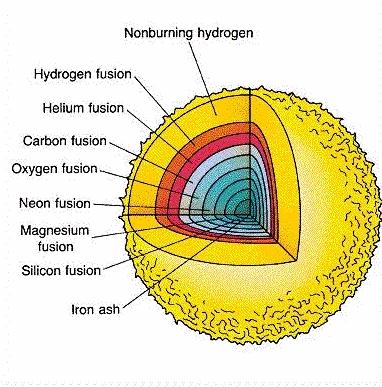
As a star ages, fusion takes place in heavier and heavier elements. Image via University of Oregon.
At this point the temperature at the star’s core may reach 3.5 billion degrees Celsius, and its “onion layers” consist of a dense iron core, surrounded by shells of silicon and sulphur, oxygen and carbon, helium and an outer shell of hydrogen. It’s incredible to realize that – to reach this late stage in its life – the star might have been in its red giant phase for a billion years! As iron cannot be burned, not even at these temperatures (fusing iron and heaver elements would require more energy than would be created), the star truly has reached the end of the road.
Once all of the core’s center has been converted to iron, a sudden and dramatic event occurs. Now, with no outward radiation pressure at all, the core completely collapses in on itself: after billions of years, gravity finally wins the stand-off!
What the core collapses into depends on the mass of the star. A star between eight and 25 times as massive as our sun will form a neutron star, while the cores of the most massive stars, more than 25 times our sun, will likely collapse into black holes.
The effect of the core’s final collapse – which takes perhaps just a second or so – is to send a shockwave right to the center, which then rebounds and propagates outward through the outer layers of the star, completely blowing it apart. Copious amounts of energy are released: hence, the brilliant event we know as a supernova explosion can be seen across the universe.
This supernova explosion is able to form all elements heavier than iron; now there is enough energy for that! For a brief period, the star can be brighter than the rest of the stars in its galaxy put together, a fiery beacon signifying the death of an ancient star. Left behind will be a neutron star or a black hole, a totally new, and final, phase in its evolution. After weeks or months, the glow of the supernova slowly fades from view, finally snuffing out altogether.
So what happens to the material flung into space in a supernova explosion, the remains of the star? It disperses gently over the eons, and its elements go toward forming new stars, new planets, perhaps even new life. All the atoms in your body were forged in the fiery hearts of ancient stars. The calcium in your bones. The iron in your blood. All were born in a huge red giant star and seeded across the universe in a supernova explosion, billions of years ago.
The two Type I sub-classes called Ib and Ic are actually similar to Type II supernovae in that all of them are produced by the core collapse of a massive star. They have their own designation because, in both cases, they lost their outer layers already before the core collapse, in a stellar wind during their red giant states, and are thus usually referred to as stripped core-collapse supernovae. Like onions already partially peeled, Type Ib has lost its first hydrogen-rich layer, and Type Ic both its hydrogen and the next helium layer, revealing the carbon-rich layer below.
The FOE
The physicists Gerry Brown and Hans Bethe devised a unit of measurement to quantify the amount of energy released in a typical Type II supernova. The measurement is stated in ergs, a unit of energy equal to 10^-7 joules. Believe it or not, the standard illustration of one erg is the amount of energy consumed by a housefly doing one pushup!
Brown and Bethe called their unit of measurement the FOE, which means ten to the power of Fifty-One Ergs; the number 10 followed by 51 zeroes. During its lifetime, the sun will emit about 1.2 FOE of energy. In other words, for the duration of 10 billion years the sun will release just a bit more energy than a Type II supernova produces in a few seconds!
For another demonstration of simply incomprehensible amounts of energy, consider this: a Type II supernova producing 1 FOE of energy may sound like a huge amount; it undoubtedly is. But now compare this to the black hole at the center of the galaxy M87, famously imaged by the Event Horizon Telescope in 2017: humans’ first image of a black hole, released in April 2019. It rotates at 90% of the speed of light. Now imagine attaching a huge dynamo to it, like that which powers a bicycle light by converting the wheel’s rotational, or kinetic, energy to electricity. How much kinetic energy would be drawn off the black hole by the dynamo? The answer is truly shocking: 10 trillion FOE. In other words, the M87 black hole’s kinetic energy is ten trillion times the energy released by a Type II supernova.
Type Ia supernovae
Quite differently (but similar to the smaller recurring nova explosions), a supernova of Type Ia will take place in a binary star system where one of the stars is a white dwarf, and the other is a companion star which the white dwarf is busy stealing matter from. A white dwarf is the superdense remnant of a smaller star (less than eight times the mass of the sun) in the last phase of its life. It is the result from the slow shrinkage, over millions of years, of a red giant star, such as Betelgeuse in the constellation Orion, recently the subject of much speculation that it was about to go supernova (it’s not). And a red giant star, in turn, is the result of a star like our sun slowly swelling up, over millions of years, when it begins to exhaust its supply of hydrogen. Our sun’s ultimate destiny is therefore to become a white dwarf, a slowly-cooling remnant of the core of our star, measuring perhaps just 0.8% of its original radius. In the case of our sun this would be a white dwarf of about 7,000 miles (11,000 km) in diameter.
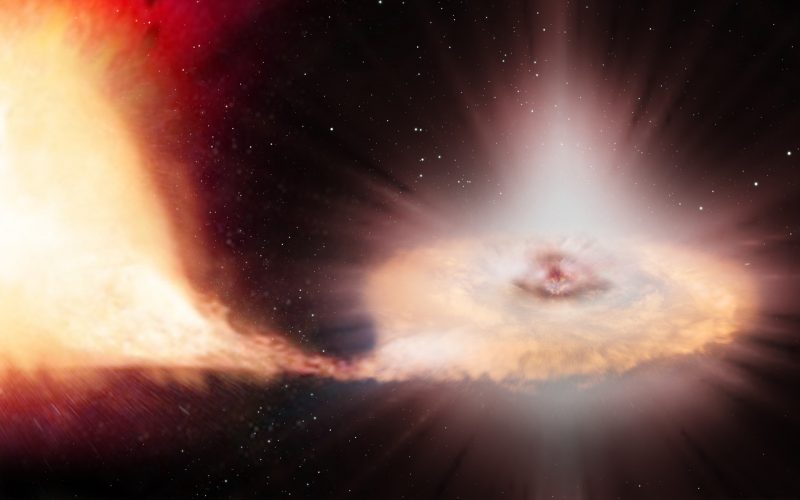
An artist’s impression of a Type Ia supernova: a white dwarf stripping its companion star of matter until it reaches the critical limit – the Chandrasekhar limit – for a supernova explosion to occur. Image via ESA/ ATG medialab/ C. Carreau.
A white dwarf star is so dense that a teaspoonful of its material would weigh about 15 tons. In its interior, all nuclear fusion processes have ceased. However – and this is the key to our supernova Ia classification – they can be reignited! If the white dwarf acquires enough material to end up with more than 1.44 times the mass of our sun, the processes can restart and lead to a runaway thermonuclear explosion and subsequent destruction of the white dwarf: a supernova. This very clear mass limit of a white dwarf is known as the Chandrasekhar Limit, named after Indian-American astrophysicist Subrahmanyan Chandrasekhar who calculated it in 1930.
How, then, can a white dwarf accumulate more mass? What happens is that the binary system contains the white dwarf and a companion, which can be any kind of star – a “normal” star, a red giant or even another (smaller) white dwarf. In the former two cases, the immense gravity of the white dwarf slowly pulls material off the companion star, accumulating it on the white dwarf’s surface until the Chandrasekhar limit is reached. Almost immediately, the resulting runaway nuclear fusion reaction blows the white dwarf apart within a few seconds: the supernova explosion. If the companion is another white dwarf, the two can merge violently, their combined mass exceeding the limit of 1.44 solar masses, again leading to a supernova explosion.
Within the Type Ia classification there are several subtypes whose exact details are still a little uncertain, but the general idea of all Type Ia supernovae is the same: a white dwarf star accumulates sufficient mass to push it over the Chandrasekhar limit, resulting in a catastrophic explosion and destruction of the star. The only exception to this is a newly discovered Type Iax supernova, which may not completely destroy the white dwarf but instead turn it into a so-called “zombie star”, keeping half its original mass. Theoretically, this could then be the cause of another supernova explosion should it merge with another white dwarf. Currently there are 30 candidates for this type of supernova which astronomers are studying.
Supernovae as useful tools
One interesting feature of Type Ia supernovae is that because of the mass limit of white dwarfs, they all explode with the same amount of energy, and hence with roughly the same brightness.
This consistency of brightness is an extremely useful way of measuring distances across the nearby universe and is known as a standard candle. If you know how intrinsically bright a Type Ia supernova is, you can measure its brightness as it appears from the Earth and thus accurately calculate its distance. It’s like seeing distant car headlights at night: you know how much light a headlight emits, so how bright it appears to you will tell you how far away it is. While we used to think that all Type Ia supernovae had exactly the same intrinsic brightness, we now know that the brightness can vary slightly. However, there is a correlation between a supernova’s luminosity and the amount of time it takes to fade from view, so its exact brightness, and hence distance, can be accurately calculated.
It was measurements of the brightness of distant supernovae which, in 1998, led a team of astronomers in the United States, Europe, Australia and Chile to a shocking discovery: the most distant Type Ia supernovae are farther away than they should be, given what was known about the age and expansion rate of the universe. This resulted in a completely unexpected realization: the universe’s expansion is actually accelerating, not slowing down over time as we had always assumed and which models predicted. Later confirmed by several subsequent studies, astronomers could not explain the accelerating universe and came up with the term dark energy to describe whatever it is that is causing it. This should not be confused with dark matter; the epithet “dark” simply means “unknown”.
To this day, the nature of dark energy is still a complete mystery, although we do know that up until around 6 billion years after the Big Bang the universe’s expansion was indeed slowing down. Then, something happened which reversed the slowdown and caused the expansion to actually accelerate. We have no idea what that event was. It’s extremely mysterious: something suddenly, as far as we can make out, changed the nature of the entire Universe. The best guess cosmologists have at the moment was that it was some sort of phase transition, an example of which is water freezing into ice and becoming something entirely different in structure and appearance. In water’s case, the event that triggers the transition, the freezing, relates to its temperature: in the case of dark energy, the event must have been something in the very structure of spacetime itself, some critical limit which had been reached. As to what that limit was, we have no idea. It may have been something entirely beyond our comprehension.
We may be decades away from understanding dark energy, although a slew of new dedicated telescopes and observation programs will seek to unravel the mystery in the upcoming years. As with the case of the search for a quantum theory of gravity, it may well take somebody of Einstein’s intellect to pull all the threads together and finally understand dark energy. However, speaking of Einstein, dark energy does seem to resemble his infamous “cosmological constant”, which was basically a mathematical trick Einstein pulled to eliminate the expanding Universe – which Einstein did not accept – from his equations. Later realizing his error, Einstein called it the greatest mistake of his life.
For their roles in leading the observations of Type Ia supernovae which resulted in the discovery of dark energy, astronomers Saul Perlmutter, Brian P. Schmidt and Adam G. Riess were awarded the Nobel Prize in physics in 2011.
Bottom line: A supernova is the cataclysmic explosion of a star at the end of its life. Supernova explosions come in different types, but all can emit more energy in a few seconds than our sun over its entire lifetime. Some supernovae outshine an entire galaxy for a brief period.
from EarthSky https://ift.tt/3pxcxvY
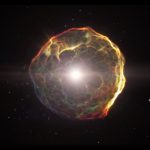
A supernova is the name given to the cataclysmic explosion of a massive star at the end of its life. It can emit more energy in a few seconds than our sun will radiate in its lifetime of billions of years.
The sky above us is strewn with alluringly beautiful remnants of ancient supernovae, that is, stars that lived out their lives and then died in these violent explosions. In a galaxy like our Milky Way, consisting of some 200 billion stars, there should be a supernova as often as every 50 years. Yet supernovae visible to the eye alone are exceedingly rare. You might – or might not – witness one in your lifetime.
EarthSky 2021 lunar calendars now available! They make great gifts. Order now. Going fast!
What we do see are supernova remnants, expanding clouds in space where stars used to be. There are many examples, both inside and outside our galaxy. The most famous supernova remnant visible from the Northern Hemisphere is called the Crab Nebula. It’s located in the direction of the constellation Taurus the Bull. The Chinese recorded witnessing the supernova in the year 1054 CE (although it had occurred 6,523 years earlier, because this star was that many light-years away). They called it a guest star and wrote that it was visible in daylight for a full three weeks, finally fading entirely from view about three months later.
Later, the Crab Nebula became famous for hosting the first known pulsar, discovered in 1967 by Jocelyn Bell Burnell, when she was a graduate student at Cambridge University in England. The Crab pulsar, as it is known, is a neutron star, the remnant of the supernova that created the Crab Nebula. Like cosmic lighthouses, pulsars give off beams of radio waves as they spin. The beams from the Crab pulsar happen to be aimed our way.
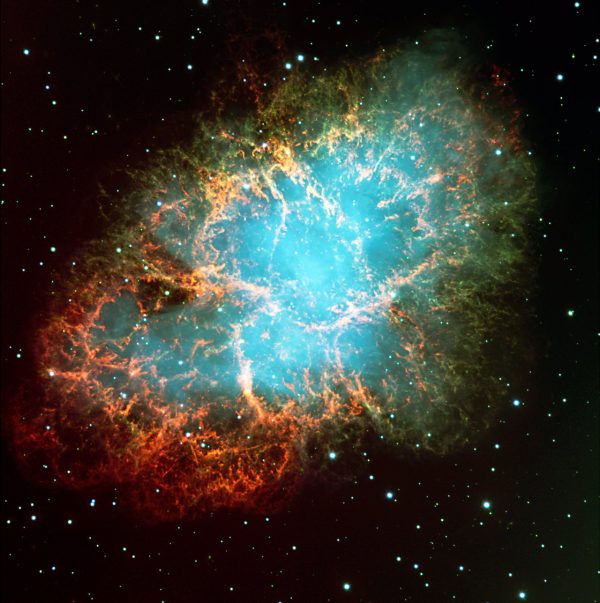
View a larger Crab Nebula image. | This photo shows a 3-color composite of the well-known Crab Nebula (aka Messier 1), as observed via the Hubble Space Telescope in 1999. It is the remnant of a supernova explosion, observed from Earth in the year 1054. It contains a neutron star near its center that spins 30 times per second around its axis. Image via HubbleSite.
So we know that supernovae are star explosions. We see examples of their aftermath in the space around us. We know that – as the star explodes outward – it also implodes, forming an exceedingly dense neutron star that might or might not appear to us on Earth as a pulsar.
But what exactly is a supernova, and what makes it explode?
Astronomers are slowly peeling away the layers of mystery surrounding these exploding stars. Their sheer unpredictability is exciting: each supernova teaches us something new. Astronomers have learned much about supernovae in just the last 50 years. A bright supernova in our Milky Way galaxy is now, statistically, long overdue. Let us hope it happens in our lifetimes, but preferably not too close!
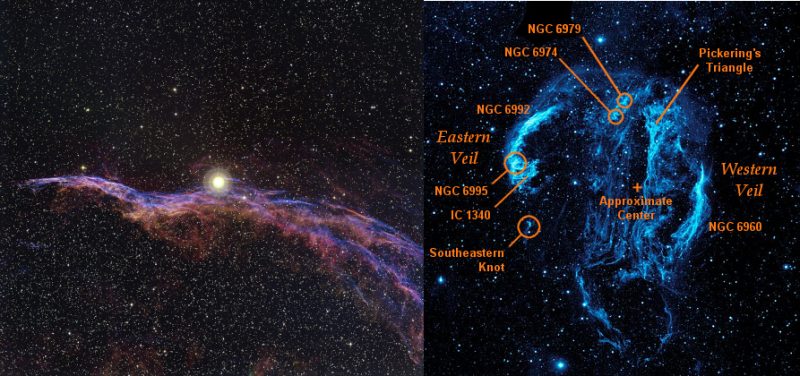
The Veil Nebula in the constellation Cygnus is another famous supernova remnant. It is the visible part of a much larger structure (the Cygnus loop) representing the remains of a star with 40 times the mass of our sun that exploded an estimated 8,000 years ago. Image (left) via T. A. Rector/ University of Alaska Anchorage/ WIYN/ NOAO/ AURA/ NSF and image (right) via NASA/ JPL-Caltech.
A supernova is a more final – and more powerful – explosion than a nova, which is the temporary flaring up of a dwarf star in a binary system. In the nova scenario, the dwarf star collects matter from its companion star. The excess mass causes the dwarf star to flare up suddenly, every now and then, to many times its normal brightness. Then it fades over months to its original brightness before the next flare-up. A supernova, on the other hand, is a much bigger and intrinsically much brighter event (hence the prefix super) where the outer layers of a star are blown explosively into space. A star that goes supernova does not return to its previous brightness and may disappear completely, leaving a an expanding supernova remnant behind.
Both novae and supernovae were once called stella novae (“new stars”), a term coined by the famous Danish astronomer Tycho Brahe in 1572. That is because both novae and supernovae can cause a “new star” to appear in our sky where none was seen before. Both flare up suddenly and – in the case of supernovae – unexpectedly, before slowly fading away entirely over weeks or months.
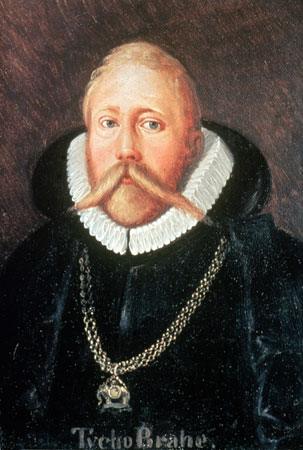
Sixteenth-century astronomer Tycho Brahe coined the term “stella nova” or new star for stars which suddenly brighten, now called novae and supernovae.
Now we know that a supernova is not a new star, but – quite the opposite – an existing one which has reached the end of its life.
And we know that novae – the less powerful flare-ups – mark the location of a star system that might flare up again.
Supernovae can have different causes, but all involve the sudden detonation of a star. Astronomers currently recognize two main types of supernovae, Type I and Type II, according to a way of classifying them devised by German-American astronomer Rudolph Minkowski and Swiss astronomer Fritz Zwicky. Hence the classification system is known as the Minkowski-Zwicky system. The classification is based on the spectra of the supernovae: that is, on their light when it is split into its component colors. Type I supernovae lack the presence of hydrogen in their spectra, while Type II supernovae display it. Type I is further divided up into three subtypes, Ia, Ib and Ic, also based on their spectra.
But maybe more exciting is the difference between the types as determined by the cause of the explosion, and here, a bit confusingly, Types II, Ib and Ic are actually the same kind of explosion, while Type Ia is a completely different creature.
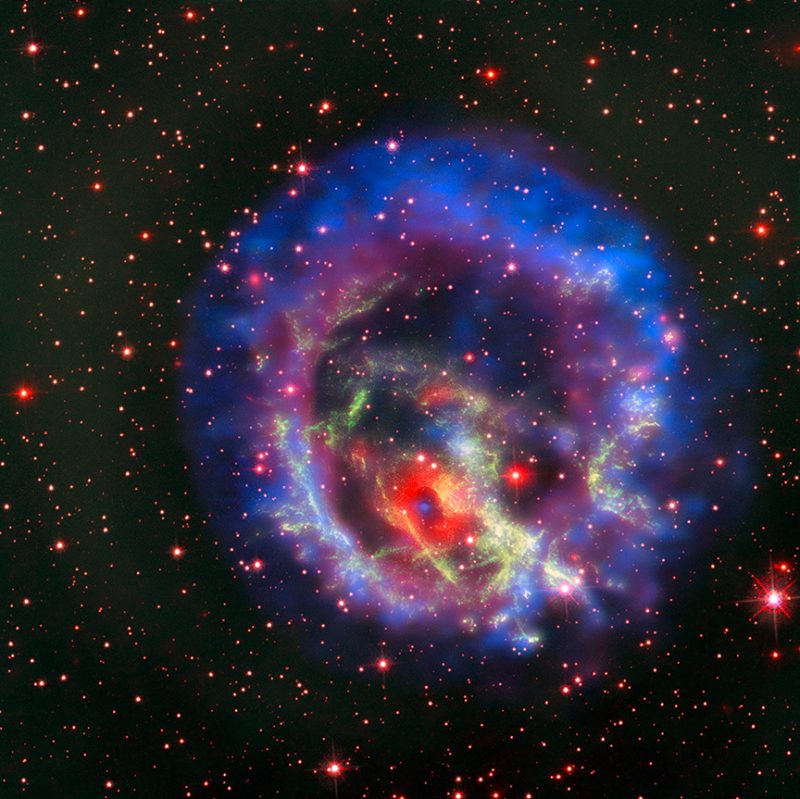
This composite image of the supernova remnant 1E0102.2-7219 contains X-ray emission measured by the Chandra telescope (blue and purple), visible light data from the optical Very Large Telescope’s (VLT) MUSE instrument (bright red), and additional data from the Hubble telescope (dark red and green). A neutron star, the ultra dense core of a massive star that has collapsed in a supernova explosion, is found at its center. Image via NASA Marshall Space Flight Center/ Flickr.
Type II Supernovae
We will start with the more common Type II, which is what people normally think of when they think of a supernova: a star exploding due to old age. Type II supernovae occur when a large star runs out of fuel, which brings it into a rapid collapse and explosion. Such a star is between eight and 40 times heavier than our sun. They are often referred to as “core collapse” supernovae because that’s exactly what happens. The star’s core suddenly – in just a few seconds – collapses in on itself.
But let’s set the scene for that cataclysmic event. For billions of years during this star’s lifetime, nuclear fusion – the process by which hydrogen is converted into helium in the star’s interior, in the process liberating huge amounts of energy, thus enabling the star to shine – had been fighting a battle with gravity. We’re not talking here about the gravitational attraction of one object toward another, but about the star’s own self-gravity. In stars, the outward-pushing radiation from fusion reactions in the star’s core is continually being countered by an inexorable inward-pushing force of gravity. It’s a duel of forces in which neither can be the victor … as long as the nuclear fusion at the core of the star is maintained, the star stays in balance.
But stars are born with a finite amount of hydrogen fuel. After billions of years (around 10 billion in the case of our sun, although our sun isn’t massive enough to produce a supernova), changes begin to take place as hydrogen is depleted. Once the core of the star completely runs out of hydrogen, nuclear fusion in the core ceases; the star has nothing left to “burn.” At that point, the star is no longer able to maintain its outward push against inward-pulling gravity. The star slowly starts to shrink. This shrinking has the effect of bringing more hydrogen from locations further out in the star into the region previously occupied by the core, sufficient hydrogen, in fact, for the nuclear fusion to resume in a shell around the star’s inner core.
However, there is something called the mirror principle, to do with the conservation of gravitational and thermal energy, which states, very simply, that if the core of a star contracts, its outer layers must expand. So the star starts to swell, expanding massively from its original size. As it does so, its outer layers cool, being further from the shell of fusing hydrogen at the core. Once the temperature cools sufficiently, convection rather than radiation becomes the dominant way the outer layers of the star are heated, and the star stops expanding. The star has become a swollen, cooler, and hence reddened, version of its former self; it is now a red giant star.
When our sun becomes a red giant, in about 5 billion years, it will expand to consume – and destroy – Mercury, Venus and possibly Earth too. This means that the sun’s diameter will increase some 115 times; it will also brighten by perhaps 3,000 times.
But – dramatic as its brightness increase may be – we’re not at the supernova stage yet, and reaching the red giant phase is not the end of the story. As the star’s core continues to shrink, temperatures inside it rise to even higher levels than before, reaching a staggering 100 million degrees Celsius. At this extreme temperature, and if the star is massive enough, it can start fusing the next element in the periodic table, that is helium, into carbon, and nuclear fusion starts up once again in its core. Temperatures rise even further, until the helium is used up, leaving a core consisting of carbon and oxygen.
The burning of its helium, however, is not a rapid process: a star eight times the mass of the sun will probably have enough helium to last 100 million years.
However, during all that time, the temperature of the core slowly increases, and when it reaches 500 million degrees, it’s hot enough for the next element to start fusing. Carbon nuclei fuse, producing sodium, neon and magnesium. All of these are burned in turn by the star; the core continues to heat up, reaching 2 billion degrees. As the temperature climbs, first silicon is formed, followed by sulphur, argon, calcium, chromium, manganese and nickel.
Each is burned in successive shells as the core continues to shrink until the star resembles an onion. Each element created in this stellar nucleosynthesis is progressively heavier – consists of larger numbers of protons, neutrons and electrons – until, eventually, one element is produced that cannot be burned: iron.
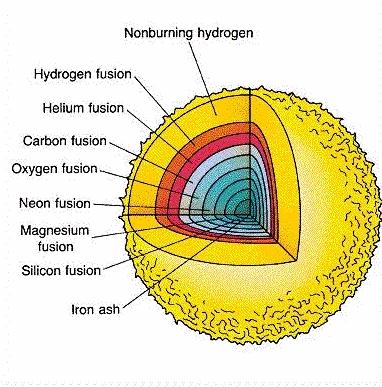
As a star ages, fusion takes place in heavier and heavier elements. Image via University of Oregon.
At this point the temperature at the star’s core may reach 3.5 billion degrees Celsius, and its “onion layers” consist of a dense iron core, surrounded by shells of silicon and sulphur, oxygen and carbon, helium and an outer shell of hydrogen. It’s incredible to realize that – to reach this late stage in its life – the star might have been in its red giant phase for a billion years! As iron cannot be burned, not even at these temperatures (fusing iron and heaver elements would require more energy than would be created), the star truly has reached the end of the road.
Once all of the core’s center has been converted to iron, a sudden and dramatic event occurs. Now, with no outward radiation pressure at all, the core completely collapses in on itself: after billions of years, gravity finally wins the stand-off!
What the core collapses into depends on the mass of the star. A star between eight and 25 times as massive as our sun will form a neutron star, while the cores of the most massive stars, more than 25 times our sun, will likely collapse into black holes.
The effect of the core’s final collapse – which takes perhaps just a second or so – is to send a shockwave right to the center, which then rebounds and propagates outward through the outer layers of the star, completely blowing it apart. Copious amounts of energy are released: hence, the brilliant event we know as a supernova explosion can be seen across the universe.
This supernova explosion is able to form all elements heavier than iron; now there is enough energy for that! For a brief period, the star can be brighter than the rest of the stars in its galaxy put together, a fiery beacon signifying the death of an ancient star. Left behind will be a neutron star or a black hole, a totally new, and final, phase in its evolution. After weeks or months, the glow of the supernova slowly fades from view, finally snuffing out altogether.
So what happens to the material flung into space in a supernova explosion, the remains of the star? It disperses gently over the eons, and its elements go toward forming new stars, new planets, perhaps even new life. All the atoms in your body were forged in the fiery hearts of ancient stars. The calcium in your bones. The iron in your blood. All were born in a huge red giant star and seeded across the universe in a supernova explosion, billions of years ago.
The two Type I sub-classes called Ib and Ic are actually similar to Type II supernovae in that all of them are produced by the core collapse of a massive star. They have their own designation because, in both cases, they lost their outer layers already before the core collapse, in a stellar wind during their red giant states, and are thus usually referred to as stripped core-collapse supernovae. Like onions already partially peeled, Type Ib has lost its first hydrogen-rich layer, and Type Ic both its hydrogen and the next helium layer, revealing the carbon-rich layer below.
The FOE
The physicists Gerry Brown and Hans Bethe devised a unit of measurement to quantify the amount of energy released in a typical Type II supernova. The measurement is stated in ergs, a unit of energy equal to 10^-7 joules. Believe it or not, the standard illustration of one erg is the amount of energy consumed by a housefly doing one pushup!
Brown and Bethe called their unit of measurement the FOE, which means ten to the power of Fifty-One Ergs; the number 10 followed by 51 zeroes. During its lifetime, the sun will emit about 1.2 FOE of energy. In other words, for the duration of 10 billion years the sun will release just a bit more energy than a Type II supernova produces in a few seconds!
For another demonstration of simply incomprehensible amounts of energy, consider this: a Type II supernova producing 1 FOE of energy may sound like a huge amount; it undoubtedly is. But now compare this to the black hole at the center of the galaxy M87, famously imaged by the Event Horizon Telescope in 2017: humans’ first image of a black hole, released in April 2019. It rotates at 90% of the speed of light. Now imagine attaching a huge dynamo to it, like that which powers a bicycle light by converting the wheel’s rotational, or kinetic, energy to electricity. How much kinetic energy would be drawn off the black hole by the dynamo? The answer is truly shocking: 10 trillion FOE. In other words, the M87 black hole’s kinetic energy is ten trillion times the energy released by a Type II supernova.
Type Ia supernovae
Quite differently (but similar to the smaller recurring nova explosions), a supernova of Type Ia will take place in a binary star system where one of the stars is a white dwarf, and the other is a companion star which the white dwarf is busy stealing matter from. A white dwarf is the superdense remnant of a smaller star (less than eight times the mass of the sun) in the last phase of its life. It is the result from the slow shrinkage, over millions of years, of a red giant star, such as Betelgeuse in the constellation Orion, recently the subject of much speculation that it was about to go supernova (it’s not). And a red giant star, in turn, is the result of a star like our sun slowly swelling up, over millions of years, when it begins to exhaust its supply of hydrogen. Our sun’s ultimate destiny is therefore to become a white dwarf, a slowly-cooling remnant of the core of our star, measuring perhaps just 0.8% of its original radius. In the case of our sun this would be a white dwarf of about 7,000 miles (11,000 km) in diameter.
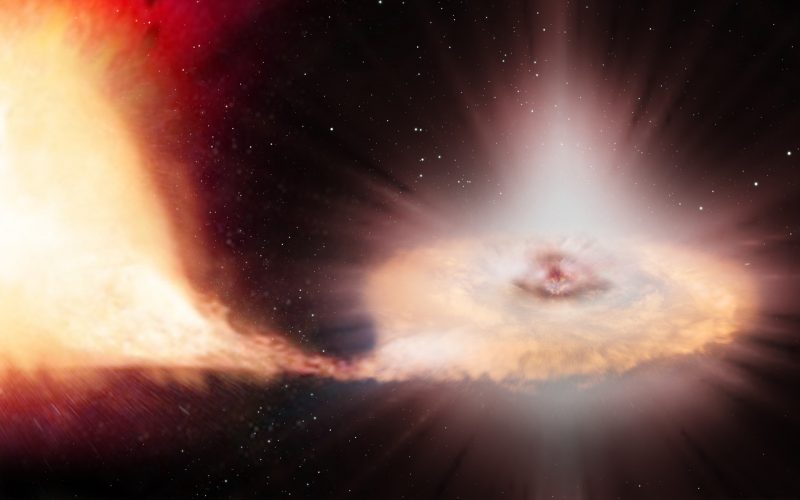
An artist’s impression of a Type Ia supernova: a white dwarf stripping its companion star of matter until it reaches the critical limit – the Chandrasekhar limit – for a supernova explosion to occur. Image via ESA/ ATG medialab/ C. Carreau.
A white dwarf star is so dense that a teaspoonful of its material would weigh about 15 tons. In its interior, all nuclear fusion processes have ceased. However – and this is the key to our supernova Ia classification – they can be reignited! If the white dwarf acquires enough material to end up with more than 1.44 times the mass of our sun, the processes can restart and lead to a runaway thermonuclear explosion and subsequent destruction of the white dwarf: a supernova. This very clear mass limit of a white dwarf is known as the Chandrasekhar Limit, named after Indian-American astrophysicist Subrahmanyan Chandrasekhar who calculated it in 1930.
How, then, can a white dwarf accumulate more mass? What happens is that the binary system contains the white dwarf and a companion, which can be any kind of star – a “normal” star, a red giant or even another (smaller) white dwarf. In the former two cases, the immense gravity of the white dwarf slowly pulls material off the companion star, accumulating it on the white dwarf’s surface until the Chandrasekhar limit is reached. Almost immediately, the resulting runaway nuclear fusion reaction blows the white dwarf apart within a few seconds: the supernova explosion. If the companion is another white dwarf, the two can merge violently, their combined mass exceeding the limit of 1.44 solar masses, again leading to a supernova explosion.
Within the Type Ia classification there are several subtypes whose exact details are still a little uncertain, but the general idea of all Type Ia supernovae is the same: a white dwarf star accumulates sufficient mass to push it over the Chandrasekhar limit, resulting in a catastrophic explosion and destruction of the star. The only exception to this is a newly discovered Type Iax supernova, which may not completely destroy the white dwarf but instead turn it into a so-called “zombie star”, keeping half its original mass. Theoretically, this could then be the cause of another supernova explosion should it merge with another white dwarf. Currently there are 30 candidates for this type of supernova which astronomers are studying.
Supernovae as useful tools
One interesting feature of Type Ia supernovae is that because of the mass limit of white dwarfs, they all explode with the same amount of energy, and hence with roughly the same brightness.
This consistency of brightness is an extremely useful way of measuring distances across the nearby universe and is known as a standard candle. If you know how intrinsically bright a Type Ia supernova is, you can measure its brightness as it appears from the Earth and thus accurately calculate its distance. It’s like seeing distant car headlights at night: you know how much light a headlight emits, so how bright it appears to you will tell you how far away it is. While we used to think that all Type Ia supernovae had exactly the same intrinsic brightness, we now know that the brightness can vary slightly. However, there is a correlation between a supernova’s luminosity and the amount of time it takes to fade from view, so its exact brightness, and hence distance, can be accurately calculated.
It was measurements of the brightness of distant supernovae which, in 1998, led a team of astronomers in the United States, Europe, Australia and Chile to a shocking discovery: the most distant Type Ia supernovae are farther away than they should be, given what was known about the age and expansion rate of the universe. This resulted in a completely unexpected realization: the universe’s expansion is actually accelerating, not slowing down over time as we had always assumed and which models predicted. Later confirmed by several subsequent studies, astronomers could not explain the accelerating universe and came up with the term dark energy to describe whatever it is that is causing it. This should not be confused with dark matter; the epithet “dark” simply means “unknown”.
To this day, the nature of dark energy is still a complete mystery, although we do know that up until around 6 billion years after the Big Bang the universe’s expansion was indeed slowing down. Then, something happened which reversed the slowdown and caused the expansion to actually accelerate. We have no idea what that event was. It’s extremely mysterious: something suddenly, as far as we can make out, changed the nature of the entire Universe. The best guess cosmologists have at the moment was that it was some sort of phase transition, an example of which is water freezing into ice and becoming something entirely different in structure and appearance. In water’s case, the event that triggers the transition, the freezing, relates to its temperature: in the case of dark energy, the event must have been something in the very structure of spacetime itself, some critical limit which had been reached. As to what that limit was, we have no idea. It may have been something entirely beyond our comprehension.
We may be decades away from understanding dark energy, although a slew of new dedicated telescopes and observation programs will seek to unravel the mystery in the upcoming years. As with the case of the search for a quantum theory of gravity, it may well take somebody of Einstein’s intellect to pull all the threads together and finally understand dark energy. However, speaking of Einstein, dark energy does seem to resemble his infamous “cosmological constant”, which was basically a mathematical trick Einstein pulled to eliminate the expanding Universe – which Einstein did not accept – from his equations. Later realizing his error, Einstein called it the greatest mistake of his life.
For their roles in leading the observations of Type Ia supernovae which resulted in the discovery of dark energy, astronomers Saul Perlmutter, Brian P. Schmidt and Adam G. Riess were awarded the Nobel Prize in physics in 2011.
Bottom line: A supernova is the cataclysmic explosion of a star at the end of its life. Supernova explosions come in different types, but all can emit more energy in a few seconds than our sun over its entire lifetime. Some supernovae outshine an entire galaxy for a brief period.
from EarthSky https://ift.tt/3pxcxvY
Aucun commentaire:
Enregistrer un commentaire